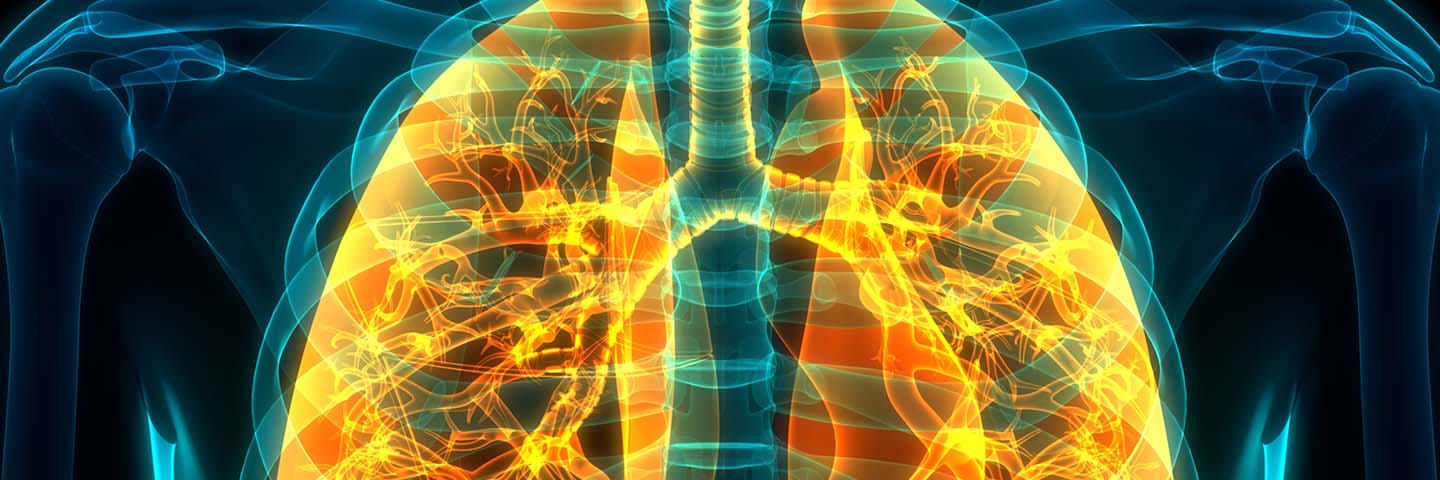
Author: Kevin Tyo, PhD
Cell Biology R&D, ATCC, Gaithersburg, MD 20877
Respiratory tract diseases stemming from inhalation exposure of toxic compounds significantly contributes to the global health burden. Traditional in vitro airway models, due to their lack of physiological relevance, are often unable to provide meaningful and accurate toxicological assessments. Advanced in vitro airway models, however, promise to provide more predictive information for use in human airway health. In this report, airway models comprising fully differentiated primary human bronchial tracheal epithelial cells (HBECs) were generated to assess toxicological response to both short- and long-term exposure from either cadmium chloride or pentamidine. Additionally, the toxicological responses from short-term exposure of compounds in airway models were compared to undifferentiated primary HBECS. Here, we show that self-constructed airway models may serve as useful tools future airway toxicity research.
Abstract
Introduction
Materials and Methods
Results
Discussion
Acknowledgements
References
Abstract
Respiratory tract diseases stemming from the inhalation of toxic compounds significantly contributes to the global health burden. Traditional in vitro airway models, due to their lack of physiological relevance, are often unable to provide meaningful and accurate toxicological assessments. Advanced in vitro airway models, however, promise to provide more predictive information for use in human airway health. In these studies, airway models comprising fully differentiated primary human bronchial tracheal epithelial cells (HBECs) incubated in 24-well plate inserts and cultured under air-liquid interface (ALI) for 4 weeks were generated. The toxicological response to short-term (24 hours) exposure to either cadmium chloride (CdCl2) or pentamidine were evaluated and compared in both airway models and undifferentiated HBECs. In addition, the toxicological response to long-term exposure (1, 2 weeks) to either compound in airway models was also explored. Changes in viability and cytokine expression was quantified and compared in both airway models and undifferentiated HBECs. Additionally, histological imaging (H&E, alcian blue, IHC) was conducted on airway models to visually assess model disruption, inflammation, and tight junction disruption. All airway models expressed dose-dependent response to both CdCl2 and pentamidine exposure, with increased cell death corresponding with increased compound concentrations. Additionally, airway models demonstrated higher resistivity to cell death compared to undifferentiated counterparts. Moreover, exposure to low concentrations resulted in increased cytokine expression relative to untreated controls. Finally, long-term exposure to CdCl2 resulted in model disruption and death, whereas pentamidine exposure demonstrated limited model disruption. These results suggest that airway models may serve as useful tools future airway toxicity research.
Download a PDF of this application note
Download nowIntroduction
The human respiratory tract is an important subject of study in a variety of fields. Respiratory infections represent the most common form of infection and act as a significant focus in disease research.1,2 Moreover, inhalation toxicity is the most prominent route of toxicity exposure, causing an estimated 7 million early deaths worldwide each year according to the World Health Organization.3,4 In pharmaceutical product development, inhalation serves as a viable of route of therapeutic administration, bypassing the first pass metabolism as well as serving as a more acceptable route of administration for patients relative to intravenous or intradermal delivery.5-7 Providing relevant models able to recapitulate the human respiratory tract for these diverse scientific fields is of critical importance for global health.
To better serve the needs of human respiratory research, 3-D-airway models have been generated to better emulate the physiological complexity of the bronchial tracheal tract.8 We seeded primary human bronchial tracheal epithelial cells (HBECs) on microporous membrane supports that were incubated while partially exposed to air, known as air liquid interface (ALI).9 Following this incubation period, the HBECs fully differentiate to generate airway models containing goblet, ciliated, and basal cell lines, providing a physiologically relevant representation of the bronchial tracheal tract. In contrast, traditional in vitro 2-D-airway models, comprising undifferentiated primary cells submerged in media, are limited in offering physiologically relevant data.10 The process of generating airway models, while lengthy compared to traditional models, can be easily performed, providing researchers with an affordable do-it-yourself model.
In the previous work “Evaluating airway ALI model fabrication methods and comparing differentiation potential of primary and hTERT-immortalized epithelial cells,” ATCC showcased an optimal method of fabricating airway models comprising human primary HBECs with consistent full epithelial differentiation using multiple primary cell lots from both ATCC and other commercial vendors. In this report, ATCC investigated the toxicological response from both 3-D airway models comprising fully differentiated primary HBECs as well as traditional 2-D in vitro models comprising freshly seeded undifferentiated primary HBECs. Two different primary cell lots were used to generate both models and were subjected to both short and long-term exposure to either cadmium chloride (CdCl2) or pentamidine. Cadmium, a metal utilized in industrial processes, is ranked 7th on the Agency for Toxic Substances and Disease Registry substance priority list as well as categorized as a substance of very high concern by EU’s European Chemical Agency due to its toxicity and risk of inhalation exposure.11,12 In contrast, pentamidine is a potent antimicrobial agent that can be administrated via inhalation and is listed in the World Health Organization list of essential medicines, however adverse side effects from its use are commonly reported.13,14 Both compounds were chosen to serve as representative substances in the fields of environmental/industrial monitoring and pharmaceutical safety. Here, various concentrations of these two compounds were utilized to compare changes in viability and inflammation from both undifferentiated cells and airway models. These studies reveal not only the differences in exposure response in both models, but also demonstrate the utility in using advanced 3-D airway models as useful tools future airway toxicity research.
Materials and Methods
Initial Cell Culture
Both lots of primary (ATCC PCS-300-010) HBECs were cultured according to ATCC’s product sheet recommendations. Briefly, all cells were first grown in ATCC complete airway epithelial growth media, consisting of ATCC Airway Epithelial Cell Basal Medium (ATCC PCS-300-030) supplemented with Bronchial Epithelial Cell Growth Kit (ATCC PCS-300-040) and 5 mL Penicillin-Streptomycin solution (ATCC 30-2300). During culturing and cell proliferation, the cell passage number for all cell lines did not exceed two. Cell growth media was replaced every other day.
For undifferentiated cell models, HBECs were passaged, and collected in growth media at a concentration of 50,000 cells/mL. 96-well plates were prepared, with each well having 0.2 mL of cell solution added (10,000 cells per well). To ensure cell attachment, plates were left at room temperature for at least one hour after seeding, followed by placement in 37°C incubation. The following day, plates underwent toxicity testing. To account for the edge effect, only the interior wells in the 96-well plates underwent testing, with the outer wells filled with 0.2 mL of Dulbecco’s Phosphate Buffered Saline (DPBS) (ATCC 30-2200).
To fabricate differentiated airway models, permeable trans-well inserts for 24-well plates with PET membrane with 0.4 µm pores (Corning cat# 353095) were placed into Costar clear 24-well multi-well plates (Corning cat# 3524) the day before cell seeding. To prevent edge effects on airway models, inserts were added to interior wells only, with outer wells filled with 2 mL DPBS. The plate inserts were then coated on the apical side with 0.3 mg/mL Collagen solution (Stemcell Technologies cat# 04902) diluted with DPBS, with plates incubating overnight at 4°C.
On the day of seeding, the collagen-coated inserts were apically rinsed twice with 200 µL DPBS, with 0.5 mL of ATCC complete airway epithelial growth media added to the basal side of wells containing trans-well inserts. HBECs were passaged, harvested, and then resuspended in growth media at a concentration of 500,000 cells/mL. The cell solution was added apically to each insert at a volume of 0.2 mL (100,000 cells per well). Following cell seeding, plates were left at room temperature for at least one hour to allow for cell attachment, followed by placement in 37°C incubation. Cells were incubated for 2-3 days until full confluency was reached. If confluency was not reach before 48 hours, both apical and basal side media were replaced with 0.2 and 0.5 mL of complete growth media respectively.
Airway Model ALI Culturing
Once reaching full confluency, both the apical and basal media were removed from each airway model, with the basal media being replaced with 0.5 mL PneumaCult ALI maintenance media (Stemcell Technologies cat# 05001) supplemented with recommended components (Stemcell Technologies cat# 07980 and 07925). In contrast, no replacement media was added to the apical side of the models, partially exposing the cells to the air. Basal media was replaced every other day with fresh complete differentiation media. Cell differentiation was observed visually via microscopy, while weekly images from airway models were collected using an EVOS FL digital microscope (Life Sciences). Following two weeks of ALI incubation, weekly apical washes with (2x) 0.2 mL DPBS were conducted to remove excess mucin from models. To confirm proper airway model formation, TEER measurements were conducted using were collected using an EVOM2 Epithelial voltmeter (World Precision Instruments). After four weeks of ALI incubation and TEER confirmation, models were considered mature and ready for toxicology testing.
Undifferentiated Cell Toxicology Testing
Following 24 hours after initial cell seeding in 96-well plates, undifferentiated HBECs were subjected to 24-hour exposure to either CdCl2 or pentamidine. Here, 16 different concentrations of CdCl2 (Sigma Aldrich cat# 20298) and pentamidine (USP cat# 1504900) were prepared in DPBS, ranging from 2.5-400 µg/mL (14-2183 µM) or 5.4-2368 µg/mL (9.3-4,000 µM) respectively. Higher pentamidine concentrations required heating solution to 37°C and vortexing vigorously to ensure complete solubility. Cell media was removed and replaced with 200 µL of respective compound concentration. Blank controls were prepared by administering 0.2mL DPBS to selected cells. Plates were placed in incubator for 24 hours following compound solution administration.
Following 24 hours incubation, 100 µL media was collected to assess IL-8 cytokine expression. Quantification of IL-8 expression was conducted using Human IL-8 ELISA kit (Invitrogen cat# KHC0081) and following the provided instructions. Viability studies were conducted by adding 100 µL CellTiter-Glo 3-D Cell Assay Reagent (Promega cat# G968B) into each sample well. Plates were incubated at room temperature for 30 minutes, followed by pipette mixing and transferring 100 µL of sample into black sided 96-well plates. Samples in wells from the black sided plates were assessed via luminescence using a SpectraMax i3x plate reader (Molecular Devices), with increased relative luminescence units (RLUs) corresponding to increased viability. All RLUs values were corrected by subtracting the media only controls, followed by converting RLU values as percent viability relative to untreated controls [% Viability = 100 × (Sample RLUs/Avg. Untreated RLUs)]. Viability changes in cell models was expressed by calculating the half maximal inhibitory concentration (IC50) using the corrected RLUs values.
Fully Differentiated Cell Model Toxicology Testing
Following four weeks of ALI, airway models were subjected to 24-hour exposure to either CdCl2 or pentamidine, using the same concentrations administered to undifferentiated HBECs. For 1- and 2-week administration, a reduced number of concentrations were used, focusing on intermediate and lower compound administration. Prior to compound administration, basal media was replaced with fresh 0.5 mL fresh differentiation media, followed by with careful rinsing of the apical side using (2x) 0.2 mL DPBS. To approximate inhalation toxic exposure, 200 µL of respective compound solutions was administered on the apical side of the airway model. For short-term exposure, airway models were incubated 24 hours after administration, followed by sample processing. For both 1- and 2-week exposure, basal media was replaced every 48 hours, with apical media replaced weekly. For sample processing, basal media was removed, followed by 100 µL apical media collection for cytokine expression. Afterwards, 100 µL CellTiter-Glo 3-D Cell Assay Reagent was added to the apical side of the membrane. Following 30 minutes of room temperature incubation, 100 µL of cell solution was transferred to black sided 96-well plates for luminescence measurements.
For histological examination, separate sets of airway models undergoing similar conditions and exposure times were prepared. Following 24 hour, 1- or 2-week exposure time, airway models were fixated and permeabilized using BD cytofix/cytoperm fixation and permeabilization solution (BD cat# BD 554722). Samples were stored in 10% ethanol at 4°C until further processing. Preserved models were paraffin embedded, sectioned, and stained with either H&E or alcian blue, followed by histological examination and imaging. Additional paraffin embedded samples underwent separate ICH staining and imaging using either Rabbit ZO-1 (Fisher Scientific cat# 40-2200) or Rabbit MUC5AC (Cell Signaling Technology Cat# 61193) antibodies.
Results
HBECs seeded in 24-well plate inserts were incubated under ALI conditions for a period of four weeks to induce epithelial differentiation. During incubation, microscopy images of airway models were taken weekly. Figure 1 illustrates the morphological changes the cells in airway models undertake during ALI, with obvious morphological changes occurring at week 2 (Figure 1C), followed by more pronounced changes occurring at week 3 (Figure 1D). Under these conditions, airway models undergo epithelial differentiation, resulting in mature airway models containing basal, goblet, and ciliated cells. These changes were observed in both primary HBEC lots as well as all airway model replicates. Moreover, TEER measurements demonstrated acceptable resistivity values and minimal variability between replicates (data not shown).
Figure 1: Representative microscopy images of primary bronchial epithelial cells under (A) 0, (B) 1, (C) 2 and (D) 3 weeks of ALI. Prolonged incubation under ALI conditions induces fully epithelial differentiation in airway models. Scale bars represent 400 µm.
The first set of viability studies were conducted to assess short-term (24 hour) exposure from CdCl2 on both undifferentiated HBECs and airway models. To visualize potential airway model disruption from CdCl2 exposure, microscopy images of mature airway models were taken 24 hours following compound administration. Figure 2 illustrates the differences in mature airway model responses from DPBS treated (blank controls), low (53.9 µM), or intermediate concentrations (795 µM) of CdCl2. Here, short-term exposure to low concentrations of CdCl2 results in no observable disruption of model integrity relative to DPBS controls, while intermediate exposure to the compound shows widespread cell death, greatly disrupting airway model integrity.
Figure 2: Representative microscopy images of airway models apically treated with either (A) 0, (B) 53.9 or (C) 795.6 µM CdCl2 for 24 Hr. No observable differences were seen between airway model blank controls and models administrated with low concentrations of CdCl2. In contrast, intermediate CdCl2 exposure results in high levels of cell death and model disruption (floating black spots). Scale bars represent 400 µm.
In addition to microscopy imaging, changes in viability were assessed by generating IC50 curves from both undifferentiated HBECs and airway models using two different primary HBEC lots (Figure 3). We observed that the undifferentiated airway models demonstrated a gradual and consistent dose-dependent decrease in viability with increased CdCl2 administration (Figure 3A), with both primary HBEC lots exhibiting IC50 values of 87.5 µM ± 10.8 and 92.5 µM ± 10.8 respectively. In contrast, airway models demonstrated higher resistivity to cell death, maintaining nearly 100% viability up to 149 µM CdCl2 exposure. However, the drop in viability in airway models was more pronounced, relative to undifferentiated HBECs (Figure 3B). The IC50 values of airway models lots 1 and 2 were calculated to be 203.1 µM ± 7.2 and 273.7 µM ± 12.3 respectively, a 2-fold increase in IC50 values relative to untreated HBECs (Figure 3C-D).
Figure 3: Changes in viability between (A) undifferentiated HBECs and (B) airway models from 24-hour exposure to CdCl2. Both undifferentiated HBECs and differentiated airway models were assessed by generating (C) IC50 curves and (D) comparing IC50 values between the two groups. Airway models demonstrated higher resistivity to cell death and IC50 values, relative to undifferentiated HBECs.
Viability measurements from short-term pentamidine exposure was also assessed. Figure 4 illustrates both the changes in viability as well as generated IC50 curves from both undifferentiated HBECs and airway models following 24-hour pentamidine administration. Similar to results from CdCl2 administration, both lots of undifferentiated primary HBECs demonstrate dose-dependent decrease in viability with increased pentamidine administration, with lots 1 and 2 exhibiting IC50 values of 60.4 µM ± 5.5 and 57.1 µM ± 13.5, respectively (Figure 4A). However, airway models exhibited significantly higher resistance to cell death relative to undifferentiated HBECs, maintaining nearly 100% viability at pentamidine concentrations 1185 µM, as well as maintaining an average viability over 10% even at the highest exposure concentration (Figure 4B). Here airway model lots 1 and 2 demonstrated IC50 values of 2,811 µM ± 201 and 2,279 µM ± 113 respectively, with IC50 values averaging over 40-fold higher than undifferentiated primary HBEC counterparts (Figure 4C-D).
Figure 4: Changes in viability between (A) undifferentiated HBECs and (B) airway models from 24-hour exposure to pentamidine. Both undifferentiated HBECs and differentiated airway models were assessed by generating (C) IC50 curves and (D) comparing IC50 values between the two groups. Airway models demonstrated significantly higher resistance to cell death and as well as over 40-fold higher IC50 values, relative to undifferentiated HBECs.
To better visualize changes in airway model structure from short-term CdCl2 exposure, histological analysis on separate sets of exposed airway models was conducted. Figure 5 shows representative images of alcian blue stained airway models incubated in either 0 (DPBS blank controls), 53.9 (low), 147.9 (intermediate), or 2183.4 µM (high) CdCl2 for 24 hours. DPBS blank controls demonstrated appropriate model morphology, showing the presence of goblet and ciliated cells (Figure 5A). Short-term exposure to low concentrations of CdCl2 resulted no noticeable differences in model morphology (Figure 5B). In contrast, intermedia CdCl2 exposure clearly results in model deterioration, with decreased model thickness and absence of cells on the membrane support (Figure 5C). Finally, high levels of CdCl2 exposure resulted in the complete degradation of the airway model, with one or two cells present on the entire membrane (Figure 5D).
Figure 5: Representative alcian blue stained images of airway models apically treated with 24-hour exposure to either (A) DPBS blank controls, (B) 53.9, (C) 147.9, or (D) 2183.4 µM CdCl2. Increased CdCl2 administration results in greater airway model disruption and cell death. Scale bars represent 20 µm.
Histological analysis was also conducted on airway models exposed to short-term pentamidine administration. Figure 6 illustrates representative images of alcian blue stained airway models incubated in either 0 (DPBS blank controls), 46.2 (low), 1,185 (intermediate), or 4,000 µM (high) pentamidine for 24 hours. Unlike CdCl2 treated counterparts, airway models demonstrated greater resistance in model degradation from short-term exposure to pentamidine, with both low and intermediate concentrations showing no observable difference in morphology relative to DPBS treated blank controls. However, slight model disruption was only observed in the highest treated (4,000 µM) pentamidine sample (Figure 6D).
Figure 6: Representative alcian blue stained images of airway models apically treated with either (A) DPBS blank controls, (B) 46.2, (C) 1,185, or (D) 4,000 µM pentamidine for 24 Hr. Compared to CdCl2 counterparts, airway models demonstrated no observable model degradation from short-term pentamidine exposure, except for the highest administered dosage of 4,000 µM, which exhibited moderate model disintegration. Scale bars represent 20 µm.
In addition to assessing changes in viability from short-term compound exposure, cytokine expression was also assessed to better examine toxicological response from both compounds. Figure 7 shows the changes in IL-8 expression in both undifferentiated HBECS and airway models from 24-hour exposure from to CdCl2. In both undifferentiated HBECs and airway models, low to intermediate administration of CdCl2 results in increased expression of the proinflammatory cytokine, compared to untreated blank controls. In contrast, models subjected to high CdCl2 administration exhibited cytokine expression levels similar to or lower than blank controls, which was attributed to cell death. In the pentamidine administered samples, high pentamidine administration resulted in little to no detectable IL-8 in both airway models and undifferentiated HBECs, whereas samples administered with low pentamidine concentrations showed lowered cytokine expression relative to blank controls (data not shown).
Figure 7: Analysis of IL-8 cytokine expression from 24-hour administration of CdCl2 to both undifferentiated HBECs and mature airway models. Values are shown as blank subtracted. Low concentrations of CdCl2 results in increased proinflammatory cytokine expression, relative to blank controls. In contrast, intermediate to high administration, results in decreased expression, due to cell death.
Following the conclusion of the short-term viability studies, airway models were subjected to one- or two-week exposure to either CdCl2 or pentamidine. Figure 8 displays the changes in viability and cytokine expression from continuous exposure to either compound. After 1-week of CdCl2 exposure, both airway model lots exhibited slightly elevated viability measurements from low exposure concentrations relative to untreated controls, probably as a function of wound healing response. In contrast, moderate long-term CdCl2 administration resulted in significant decrease in airway model viability. Additionally, 2-week exposure results exhibited similar trends, with low CdCl2 concentrations demonstrating near equivalent viability response relative to untreated controls (Figure 8A). Viability measurements taken from long-term pentamidine administration resulted in similar trends to CdCl2 exposure, with low pentamidine concentrations demonstrating comparable viability response to blank controls whereas long-term moderate pentamidine exposure resulted in decrease viability (Figure 8B). Cytokine expression was also assessed using apical washes collected at the end of compound administration (Figure 8C). Similar to short-term testing, airway models demonstrated elevated levels of cytokine expression to low cadmium administration following 1 week incubation relative to untreated controls, whereas moderate cadmium administration results in low cytokine expression, attributed to cell death. There was no observable trend between increased cytokine expression to increased compound administration following 2-week administration, which was attributed to suboptimal cytokine sample collection from long-term studies (data not shown).
Figure 8: Changes in viability in airway models exposed to either (A) CdCl2 or (B) pentamidine for 1 and 2 weeks, (C) as well as cytokine expression following 1 week administration of CdCl2.
Parallel with long-term viability measurements, histological assessments were also conducted on separate airway model samples. Figure 9 shows representative images of alcian blue stained airway models treated with either low to moderate administrations of either CdCl2 or pentamidine for one week. Increasing CdCl2 administration during this period results in progressively deteriorating airway model integrity. Pentamidine exposure showed a similar, albeit a more gradual trend, with model integrity being better preserved from long-term pentamidine exposure. These trends were observed in two week administered samples as well (data not shown).
Figure 9: Representative alcian blue stained images of airway models apically treated with either (A) 0, (B) 14.0, (C) 53.9, or (D) 795.6 µM CdCl2, or (E) 0, (F) 46.2, (G) 156, (H) 1185 µM pentamidine for 1 week. Long-term exposure from low or moderate concentrations of CdCl2 results in airway model disruption. In contrast, airway model integrity is better preserved from long-term pentamidine exposure. Scale bars represent 20 µm.
Finally, separate IHC staining and imaging was conducted to visualize potential ZO-1 protein disruption from long-term CdCl2 exposure as well as increased MUC5AC expression short-term pentamidine administration (Figure 10). Representative IHC-stained images of airway models stained for ZO-1 protein demonstrated that decreased ZO-1 expression is correlated to increased CdCl2 administration, relative to blank controls (Figure 10A-C). In contrast, no observable correlation was found between with increased MUC5AC expression from increasing pentamidine administration (Figure 10D-F). This result was attributed to the preparation of histology samples, which may have resulted in the washing and removal any excess mucin from the airway model samples.
Figure 10: Representative ICH-stained images of (A) untreated control airway models, airway models exposed to (B) 14.0, or (C) 147.9 µM CdCl2 for 2 weeks. ZO-1 protein expression is shown as red with DAPI control in blue. ZO-1 protein disruption is associated with increased CdCl2 exposure. Representative ICH-stained images of airway models treated with (D) 0, (E) 9.1, (F) 46 µM pentamidine for 24 Hr. MUC5AC expression shown as red with DAPI control in blue. There was no correlation with increased MUC5AC expression with increasing pentamidine administration.
Discussion
In these studies, self-constructed airway models comprising fully differentiated HBECs were fabricated for use in toxicological studies. Here, the toxicological response between airway models and freshly seeded undifferentiated HBECs from short-term administration of two different compounds were compared. Moreover, this study also examined the variation between models comprising two separate primary cell production lots. Finally, long-term exposure studies of airway models were also explored.
During these studies, compounds were administered to the apical side of airway models to better approximate the physiologically relevant exposure of the human lung to toxic compounds. We observed that airway models subjected to short-term CdCl2 or pentamidine exposure exhibited average IC50 values of 238 and 2545 µM, respectively. To our knowledge, no relevant study has yet been conducted to assess changes in viability in airway models from 24-hour exposure to pentamidine. In contrast, a previous study testing airway models to short-term CdCl2 exposure estimated IC50 to be within a range 100-300 µM, in agreement to our findings.15 However, the referenced study administered CdCl2 on the basal side. This difference may not affect airway models comprised of only HBECs; however, co-culture models may not respond in a physiologically relevant manner to compound administration through basal media.
Short-term cytokine results aligned with our expectations, with low-moderate CdCl2 administration results in higher cytokine expression relative to blank controls, whereas high compound fails to elicit higher cytokine expression due to greater cell death. In contrast, pentamidine administration failed to induce increased cytokine expression. Previous in vitro studies showed that pentamidine exposure inhibits IL-8 expression, which was observed in this study as well.16 During long-term testing no trend was observed during 2-week incubation, which was attributed to cytokine sample loss when basal media was replaced during the study. Although basal media replacement is vital in sustaining airway model viability, any media or solution incubated with airway models should be collected and assessed during the study. Long-term viability measurements were unaffected to this incident due to directly assessing primary cell metabolism.
These studies showcase how self-fabricated airway models can be utilized to provide data that's more physiologically relevant to the human airway model. This was clearly demonstrated when comparing the response from both undifferentiated HBECs and airway models from pentamidine administration. Moreover, the seeding of additional cells, such as fibroblasts, dendritic, or endothelial cells are possible using this airway model fabrication method and may offer additional insights utility in toxicology studies.
Acknowledgements
Download a PDF of this application note
Download nowReferences
- GBD 2016 Lower Respiratory Infectious Collaborators. Estimates of the global, regional, and national morbidity, mortality, and aetiologies of lower respiratory infections in 195 countries, 1990-2016: a systematic analysis for the Global Burden of Disease Study 2016. Lancet Infect Dis, 2018. 18(11): p. 1191-1210.
- Wang, X., et al., Global burden of acute lower respiratory infection associated with human metapneumovirus in children under 5 years in 2018: a systematic review and modelling study. Lancet Glob Health, 2021. 9(1): p. e33-e43.
- Burnett, R., et al., Global estimates of mortality associated with long-term exposure to outdoor fine particulate matter. 2018. 115(38): p. 9592-9597.
- Organization, W.H. 9 out of 10 people worldwide breathe polluted air, but more countries are taking action. 2018; Available from: https://www.who.int/news/item/02-05-2018-9-out-of-10-people-worldwide-breathe-polluted-air-but-more-countries-are-taking-action.
- van den Berg, A.A., et al., Intravenous or Inhaled Induction of Anesthesia in Adults? An Audit of Preoperative Patient Preferences. 2005. 100(5): p. 1422-1424.
- Stewart, K.D., et al., Preference for pharmaceutical formulation and treatment process attributes. Patient Prefer Adherence, 2016. 10: p. 1385-99.
- Losi, S., et al., The role of patient preferences in adherence to treatment in chronic disease: a narrative review. Drug Target Insights, 2021. 15: p. 13-20.
- Cao, X., et al., Invited review: human air-liquid-interface organotypic airway tissue models derived from primary tracheobronchial epithelial cells-overview and perspectives. In Vitro Cell Dev Biol Anim, 2021. 57(2): p. 104-132.
- Rayner, R.E., et al., Optimization of Normal Human Bronchial Epithelial (NHBE) Cell 3-D Cultures for in vitro Lung Model Studies. Sci Rep, 2019. 9(1): p. 500.
- Bérubé, K., et al., Human primary bronchial lung cell constructs: the new respiratory models. Toxicology, 2010. 278(3): p. 311-8.
- Faroon, O., et al., Toxicological Profile for Cadmium. 2012, Agency for Toxic Substances and Disease Registry (US), Atlanta (GA).
- Nordberg, G.F., et al., Risk assessment of effects of cadmium on human health (IUPAC Technical Report). 2018. 90(4): p. 755-808.
- Scheld, M.W., B. Wispelwey, and R.D. Pearson, Pentamidine: A Review. Infection Control & Hospital Epidemiology, 1991. 12(6): p. 375-382.
- Monk, J.P. and P. Benfield, Inhaled Pentamidine. Drugs, 1990. 39(5): p. 741-756.
- Cao, X., et al., Tight junction disruption by cadmium in an in vitro human airway tissue model. Respir Res, 2015. 16(1): p. 30.
- Van Wauwe, J., et al., The inhibitory effect of pentamidine on the production of chemotactic cytokines by in vitro stimulated human blood cells. Inflamm Res, 1996. 45(7): p. 357-63.